An Ecosystem for Self-Growing Digital Twin Supporting Smart-City-as-a-Service
~Reliable Data Caching Technology in Information-Centric Wireless Networks~
目 次
- Ⅰ. Summary
- Ⅱ. Introduction
- Ⅲ. Research and Development Items
- Ⅲ.A. Overview
- Ⅲ.B. Research plan
- Ⅲ.C. (2-d-ⅰ) Implementation, development, and evaluation of ICWSNs (for terrestrial network)(FY2022)
- Ⅲ.D. (2-d-ⅱ) Implementation, development, and evaluation of ICWSNs (for non-terrestrial network)(FY2023)
- Ⅲ.E. (2-d-ⅲ) Establishment of elemental technology for improving the reliability of ICWSNs (FY2023)
- Ⅲ.F. (2-d-ⅳ) Cross-sectional demonstration and evaluation of integrated non-terrestrial ICWSNs (FY2024)
- Ⅲ.G. (2-d-ⅴ) Demonstration and evaluation of reliable ICWSNs (FY2024)
- Ⅲ.H. (3-b-①-ⅰ) Prototyping: 3D sensor system (fundamental design) (FY2022)
- Ⅲ.I. (3-b-①-ⅱ) Prototyping: 3D sensor system (test operation) (FY2023)
- Ⅲ.J. (3-b-①-ⅲ) Prototyping: 3D sensor system (proof-of-concept experiments with horizontal scalability) (FY2024)
- Ⅳ. Findings and Results
- Ⅳ.A. (2-d-ⅰ) Implementation, development, and evaluation of ICWSNs (for terrestrial network) (FY2022)
- Ⅳ.B. (2-d-ⅱ) Implementation, development, and evaluation of ICWSNs (for non-terrestrial network)(FY2023)
- Ⅳ.C. (2-d-ⅲ) Establishment of elemental technology for improving the reliability of ICWSNs (FY2023)
- Ⅳ.D. (2-d-ⅳ) Cross-sectional demonstration and evaluation of integrated non-terrestrial ICWSNs (FY2024)
- Ⅳ.E. (2-d-ⅴ) Demonstration and evaluation of reliable ICWSNs (FY2024)
- Ⅳ.F. (3-b-①-ⅰ) Prototyping: 3D sensor system (fundamental design)(FY2022)
- Ⅳ.G. (3-b-①-ⅱ) Prototyping: 3D sensor system (test operation)(FY2023)
- Ⅳ.H. (3-b-①-ⅲ) Prototyping: 3D sensor system (proof-of-concept experiments with horizontal scalability)(FY2024)
- Ⅴ. Conclusion
- Acknowledgement
- Research Outcomes
Ⅰ. Summary
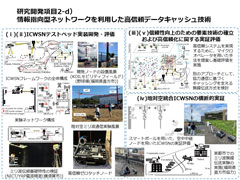
(click to zoom)
We have completed the goals we set out as folows.
[Goals]
- Data caching technology for ICN using blockchain will be developed.
- Non-terrestrial ICWSN will be developed and demonstrated.
- Reliable data caching technology for ICWSNs will be developed.
- Practical solution to solve the problems that are expected to occur when the system is actually deployed in a smart city will be investigated.
[Achievements toward the goals]
- Data caching technology for ICWSNs using blockchain have been developed.
- ICWSN testbed have beeen developed and implemented.
- Elemental technology for improving the reliability of data collection in ICWSNs have been developed, and it have been evaluated in a realistic environment.
- Ait-to-ground integrated ICWSN have been constructed.
- Demonstration and experiment have been conducted to identify practical issues that cannot be known through just paperwork.
- Reliable zero-touch sensor node have been implemented and developed, and the experiments have been conducted to evaluate the effectiveness of the proposed system at two locations: the KOIL Mobility Field and the area around Fukuoka University.
Ⅰ. Introduction
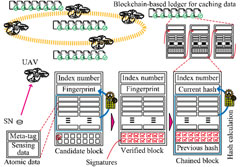
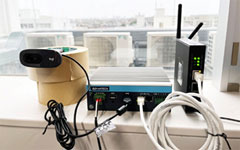
In many studies of introducing information-centric networking (ICN) into wireless communication systems, the main focus is on efficiently providing content data to users. In other words, the most common approach is to cache data from the content server on the base station and then distribute the data to users efficiently. In the information-centric wireless sensor network (ICWSN), which underpins future Internet of Things (IoT) systems in the next-generation wireless communication system (beyond the fifth generation (B5G)), it is necessary to consider the uplink from the wireless sensor network (WSN) to the base station, so its protocol design has potential for improvement. In addition, many related studies have been done to evaluate its performance using computer simulations; we believe it is important to evaluate ICWSN in realistic environments and scenarios using actual hardware devices. Therefore, in the research and development items that we are assigned as co-proposers in this project, we will establish the elemental technology for improving the reliability of data collection using ICWSN, implement a prototype system, and conduct field demonstrations.
In order to make communication networks more reliable, they should be designed to ensure that service is not disrupted even if a fault occurs in a node. In 5G/B5G, network slicing technology is used to support multiple application use cases. In order to ensure reliable data transfer between multiple wireless nodes, it is necessary to develop a new wireless network technology. Ultra-reliable and low latency communications (URLLC) for 5G wireless transmission aims to achieve a packet availability of five nines (99.999% or more). Besides, the reliable data caching technology here responds to requests from the data requestor during an effective period (sensing data is valid for meaning).
However, the wireless nodes that makeup WSNs are limited in terms of battery life and computing power, and their wireless communication environment is inferior to that of wired networks. Therefore, it is unrealistic to expect that the ICN technology developed for wired networks is applicable to WSNs. In other words, even if the topology of the communication network is guaranteed to be connected, there might be cases where communication is not possible, and even if communication is possible, communication services may be disrupted if the link is momentarily interrupted due to the communication environment. Another aspect of reliable data caching technology is that it must also be secure. When transmitting data securely over a wireless link, it is common to establish a secure wireless link between the transmitter and receiver and then transfer the data over this secure link. In this situation, if we introduce ICN into WSN, we can protect the data itself directly instead of protecting the wireless communication channel. As a result, there are advantages in terms of reducing the overhead of processing to establish a secure wireless link and the processing required to re-establish the link when it becomes unstable. In addition, since it will also become a data transmission with privacy-sensitive data via the same wireless communication channel, there is also the advantage that the mechanism for achieving secure wireless links can be omitted.
Thanks to the advantages of ICN, we will develop elemental technology for a reliable ICWSN in which neighboring nodes complement each other, even if the wireless network is dynamically variable due to node joining/leaving, node movement, or propagation-environment changes. Specifically, we plan to introduce the secure caching scheme using the blockchain we developed in the previous study, as well as a method that can restore data even if some frames do not arrive at the receiver using error-correcting codes. Furthermore, in the blockchain-based system, we have already begun considering a new method of consensus based on a voting process (Fig.1). In this system, mobile entities, such as pedestrians, small vehicles, and unmanned aerial vehicles (UAVs) not only collect data but also act as validators, and the validation of committed blocks is approved based on voting by the participants themselves. Therefore, unlike other blockchains (e.g., Bitcoin and Ethereum), the blocks can be verified without large amounts of calculation, like the proof-of-work (PoW) method, which is effective for the hardware limitations in ICWSNs. We plan to achieve this project's goal by integrating the methods we have studied.
This research item aims to improve the reliability of data collection in WSNs using ICN and blockchain technology. Through previous studies, we developed a sophisticated fusion of ICN and blockchain and made it into ICNWSNs. Waseda University, which specializes in ICN, and Gaiax, which specializes in blockchain (and its business development), are both participating, and further development and deployment of ICWSN in society can be expected. In addition, our overseas partner, Professor Andrea Detti of Italy, has also been studying ICN for many years. In collaboration with these organizations, we plan to enhance the technology of ICWSN and also make standardization proposals to strengthen the international competitiveness of this field (Waseda University will take the lead in making standardization proposals).
In the development of the testbed, we have constructed an ICN wireless communication test environment using the MIC-710AIX from Advantech Japan as an industrial-embedded computer using LTE connections, and we have been conducting ongoing experiments. As this prototype can be expanded to enable various air interfaces, it is also compatible with millimeter waves, sub-gigahertz waves, and microwaves, which will be used in the field experiments in this project. As we can see, although we still need to make some adjustments to the connectivity and device customization in order to verify the system in a practical field, we have already prepared a prototype that is designed to be evaluated, and we are currently working on operational verification in a laboratory environment (Fig.2).
Ⅲ. Research and Development Items
In this section, we describe reliable ICWSN system in the R&D items (2-d) and (3-b-①).
Ⅲ.A. Overview
In the R&D items (2-d) and (3-b-①), we aim to establish the elemental technologies that contribute to the “reliability” required for B5G. For the existence of attackers, we consider the design of a blockchain network system that guarantees the reliability of data, malicious poison data, and attacks on artificial intelligence (AI) models. In particular, we develop a reliable data caching technology for ICWSNs. The KOIL Mobility Field (Kashiwa City, Chiba Prefecture) is used as a field demonstration site for the prototype implementation. As communication infrastructure, low-power wide-area network (LPWAN), Wi-Fi, and Terragraph's millimeter wave (mmWaves) mesh network are available in the KOIL Mobility Field. In addition, it has been collecting information, such as images from cameras, temperature, humidity, noise, and sunlight levels. However, it does not collect 3D sensing data or meteorological information (e.g., wind speed and direction) in the sky, which is essential for the city-as-a-service platform in a future city, and it does not distribute these data or build a digital twin model. Therefore, during this project, the effectiveness of the ecosystem is to be verified to seamlessly share and distribute the latest urban digital data in the Kashiwa-no-ha Smart City.
Ⅲ.B. Research plan
In FY2022, we will design and develop prototypes of the elemental technologies for each R&D items. In particular, we will carry out fundamental experiments and purchase the equipment necessary for field testing in FY2024.
In FY2023, we will evaluate the effectiveness of the method we tested and examined in FY2022 in a laboratory environment. Based on the results, we will consider improving the individual technologies of each R&D item for field verification in FY2024. For each R&D item, the developed systems that are designed based on the 3D sensor system and smart pole, as well as the fundamental characteristics, are evaluated in the KOIL Mobility Field. The results from these activities are then reflected in each R&D item, and insufficient functional requirements are identified and used for method improvements. We will ensure that the prototyping process is without any delays, and we will use the evaluation results and findings obtained to ensure that the prototype is implemented by the end of FY2023.
In FY2024, to realize an ecosystem that enables the digital twin that supports the city-as-a-service to grow sustainably, we will continue to upgrade and expand the functions of each sensor system developed in FY2023 and integrate the functions. Finally, the smart sensor system will be implemented, and field testing will be carried out at the KOIL Mobility Field. Through this field demonstration, we will identify how the digital twin is established by simply turning on the power of the smart sensor system and how the IoT network connects each device and the data distribution.
Ⅲ.C. (2-d-ⅰ) Implementation, development, and evaluation of ICWSNs (for terrestrial network)(FY2022)
In the R&D item (2-d-i), we will develop reliable data caching technology for ICWSNs. In the future, it is expected that IoT devices on the ground and in the air will automatically form self-growing ad hoc WSNs. Here, we will tackle the challenge of realizing a reliable WSN using ICN and its caching function.
Ⅲ.D. (2-d-ⅱ) Implementation, development, and evaluation of ICWSNs (for non-terrestrial network)(FY2023)
We will implement, develop, and evaluate an experimental testbed for constructing an ICWSN that includes IoT devices in the air by interconnecting the ground-based devices that were realized in the R&D item (2-d-i). In addition, we will implement and develop a device that can operate stably over the long term as a node necessary for building and evaluating reliable WSNs. The evaluation will be carried out at two locations: the KOIL Mobility Field and the Fukuoka University area, and it will realize a reliable WSN using ICWSN technology.
Ⅲ.E. (2-d-ⅲ) Establishment of elemental technology for improving the reliability of ICWSNs (FY2023)
In many studies that introduce ICN into wireless communication systems, the main focus is providing users with content data efficiently. In other words, the key concept is to cache data from the content server on the base station (BS) and then distribute it to users efficiently. On the other hand, in the ICWSN supporting future IoT systems in B5G, it is necessary to consider the uplink from the WSN to the BS, so there remains potential for improvement in the protocol design. In addition, in most related studies, the evaluations were conducted using computer simulations. Therefore, we believe that the evaluation of ICWSN in a practical environment and scenario using hardware devices is meaningful. In this R&D item, we will establish the elemental technology for improving the reliability of data collection in ICWSNs. In order to make communication networks more reliable, it is necessary to design them to ensure that even if a fault occurs in a node, it will not affect the service. In this section, we consider reliable data caching systems to be those that can respond to requests from data acquirers during the period when the sensing data is valid and meaningful.
Ⅲ.F. (2-d-ⅳ) Cross-sectional demonstration and evaluation of integrated non-terrestrial ICWSNs (FY2024)
The exploration of higher frequency spectrum to drive the next-generation wireless communication systems is an urgent task, and Terragraph, an mmWave-based communication system, is one of the solutions to this challenge. In the R&D item (2-d-ii), we conducted a fundamental evaluation of the integrated ground-to-air ICWSN using an aerial relay node carried out basic measurements of radio wave propagation, and conducted a long-term operation test for 60 GHz-band waves. Here, on the basis of the long-term operational testing in FY2023, we will continue the above test operation and verify the influence of weather conditions (e.g., rainfall and snowfall) and ambient conditions on the wireless communication system. This R&D item aims to clarify practical issues that cannot be identified from the paperwork, and we will consider the solutions for these issues. We believe that the results of this investigation can be used to improve the reliability of the system.
For the horizontal development of the ICWSN system, we will evaluate the characteristics of multiple aerial nodes in various environments. The experiment includes collaboration with other co-proposers, and the development of the ICWSN system will be examined from different perspectives. The results can provide significant knowledge for future research and development.
Ⅲ.G. (2-d-ⅴ) Demonstration and evaluation of reliable ICWSNs (FY2024)
In the B5G, the ICWSN that will support future IoT systems also needs to consider the uplink from the WSN to the BS, so the protocol design has considerable margins. In other related studies and development of the ICWSN, there have been many investigations and evaluations using computer simulations, but the significance of evaluations in practical environments and scenarios using hardware devices is considerable. Therefore, in this section, we will utilize the knowledge gained from the testbed development conducted in the R&D Item (2-d-i) and (2-d-iii) to implement and develop a reliable zero-touch node device, and we will also experiment with two locations: the KOIL Mobility Field and the Fukuoka University area.
Ⅲ.H. (3-b-①-ⅰ) Prototyping: 3D sensor system (fundamental design)(FY2022)
Before conducting field tests using the prototype device, we will implement and develop a portable-type node. The main purpose of this trial is to conduct long-term operational testing on a laboratory scale, including testing the communication between the sensors and wireless modules on the specified node. In addition, a prototype network will be constructed in a practical test field. Through these activities, we will identify issues to be tackled before the experimental evaluation using a testbed with the results of the R&D item (2-d) that we plan to conduct in the next fiscal year and beyond, and we will also consider the test operation of the prototype.
Ⅲ.I. (3-b-①-ⅱ) Prototyping: 3D sensor system (test operation)(FY2023)
A prototype network will be constructed and tested in a practical field. In addition, we will identify issues prior to the experimental evaluation using the test bed that has introduced the R&D item (2-d), and we will consider the test operation of the prototype device. In collaboration with the R&D item (2-d-ii), the test operation will not only be carried out at the KOIL Mobility Field but also at a location near Fukuoka University, where some of the functions will be replicated so that a multidimensional operational evaluation can be performed.
Ⅲ.J. (3-b-①-ⅲ) Prototyping: 3D sensor system (proof-of-concept experiments with horizontal scalability)(FY2024)
Based on the results in the R&D items (3-b-①–ii) and in collaboration with the R&D items (2-d-iv) and (v), we will conduct a demonstration evaluation based on the basic design carried out in the R&D item (2-d-iii). We also plan to include demonstration evaluations in collaboration with other organizations, considering horizontal development. Based on the findings of this section, we will also consider what kind of solutions can be provided for users when the developed ICWSN system is deployed in a smart city. During the development of the ICWSN system, it is helpful to conduct experiments and evaluations in real cities as a test field in order to promote the horizontal deployment of the developed ICWSN system. Therefore, we will work with Nogata City in Fukuoka to provide a demonstration field for testing and operating the development system.
Ⅳ. Findings and Results
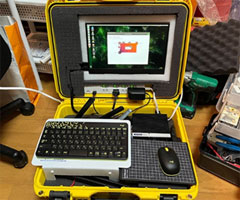
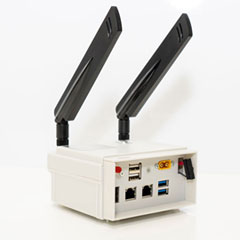
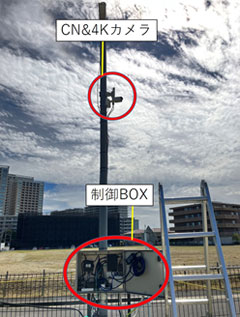
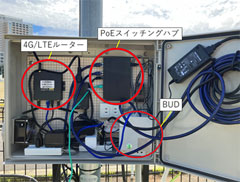
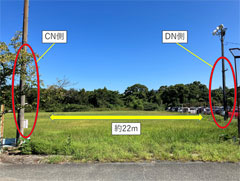
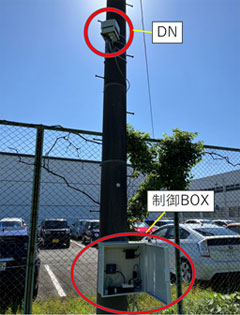
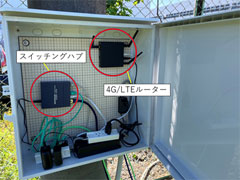
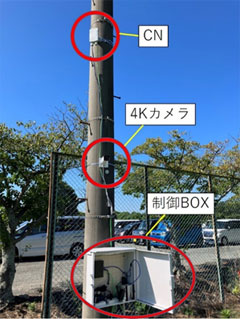
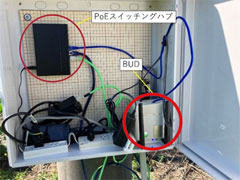
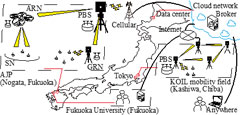
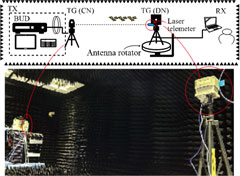
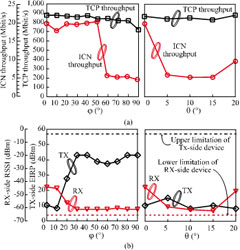
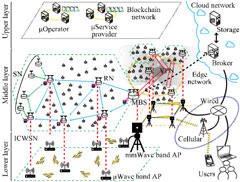
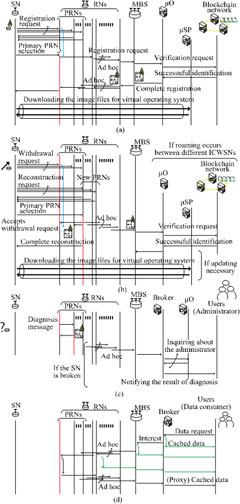
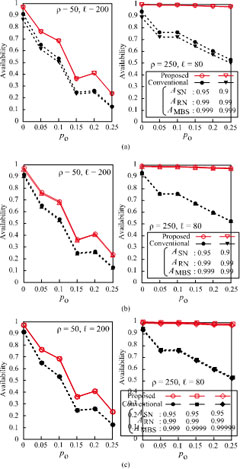
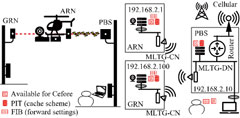
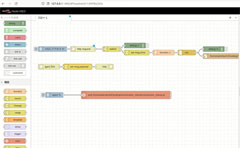
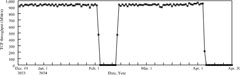
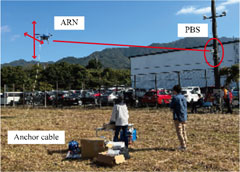
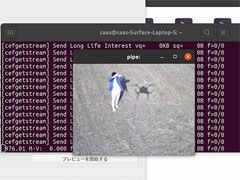
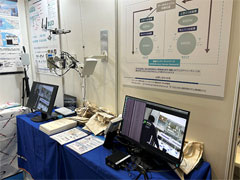
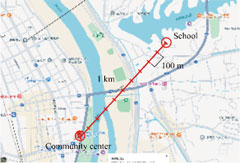
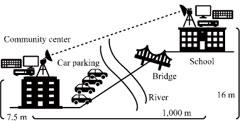
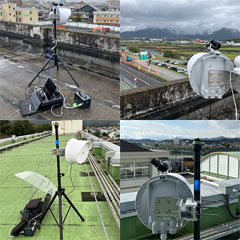
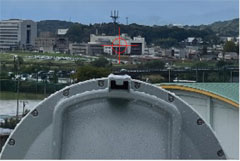
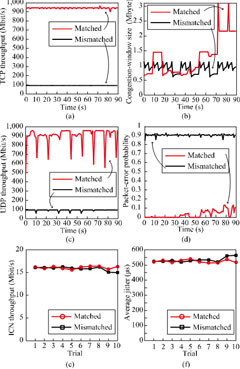
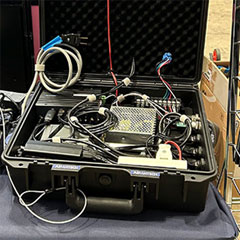
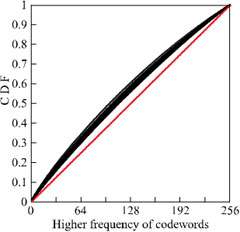
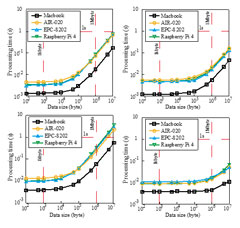
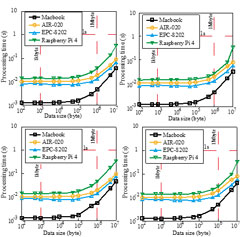
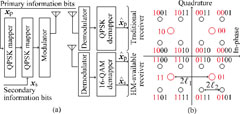
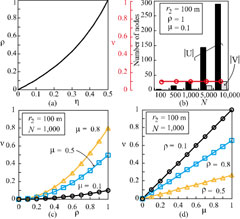
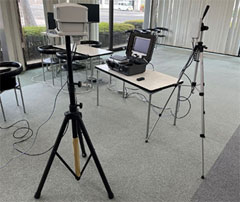
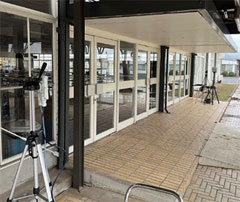
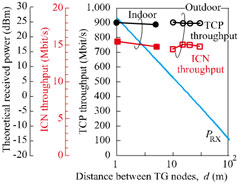
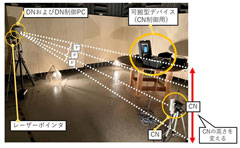
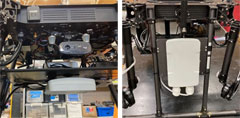
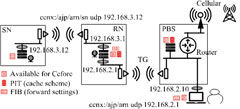
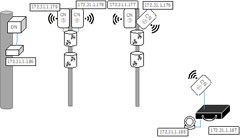
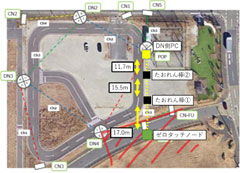
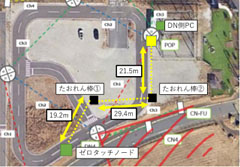
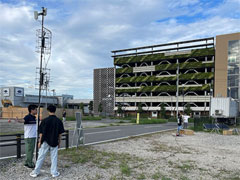
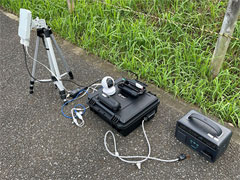
Ⅳ.A. (2-d-ⅰ) Implementation, development, and evaluation of ICWSNs (for terrestrial network)(FY2022)
In the R&D item, we aim to establish the elemental technology contributing to the “reliability” that B5G requires. Specifically, we consider the participation and withdrawal of participants to occur frequently, and we investigate reliable data caching technology for ICWSNs. In particular, WSNs have significant hardware limitations in battery and processing power compared to wired networks. It can provide a reliable data cache even within such limitations. IoT devices that exist on the ground and in the air can be considered to automatically form ad hoc WSNs and be self-growing. We implement and evaluate the development of the ground-deployed, portable node as a testbed device necessary for the ICWSN field experiment.
We developed the devices to evaluate a reliable ICWSN, interconnecting them with the ground-based devices, as shown in Fig.3. We implemented the customizations and portability required to experiment in the KOIL Mobility Field. We deployed a prototype device and identified the issues related to actual deployment using it through fundamental evaluation. In the device, we replaced the onboard computer with the AIR-020X, which is a superior model compared to the MIC-710AIX, and we used Terragraph as the air interface.
Through the R&D activities here, we found that the current device could not satisfy the technical requirements in terms of weight and size when it was deployed in the sky, despite its usability on the ground. Therefore, we developed a test device that was customized to be used in this proof-of-concept experiment as an alternative device based on the Advantech Brain Unit for Drone (BUD), which was used in drone applications and had previous experience, as shown in Fig.4. In particular, it is specified as a unit that can operate on power supplied from a lithium-ion battery.
Ⅳ.B. (2-d-ⅱ) Implementation, development, and evaluation of ICWSNs (for non-terrestrial network)(FY2023)
In the R&D item (2-d-i), we have developed hardware devices for the ground-deployed, portable node as a testbed device necessary for the ICWSN field experiment. However, it is expected that IoT devices on the ground and in the air will automatically establish self-growing based on ad-hoc networks. Therefore, we constructed a test field and the nodes for evaluating a non-terrestrial ICWSN connected to the implemented terrestrial devices in the R&D item (2-d-i). The test field was prepared in the KOIL Mobility Field and the area around Fukuoka University (the baseball field at Advantech Nogata Office in Nogata City, Fukuoka). We have prepared for the experiment.
Figures 5–6 show an overview of the facilities located in the KOIL Mobility Field. The power supply was obtained by connecting the commercial power supply in the KOIL Mobility Field to the control box. For the internet access, we have two connections, including a backup. The main connection is via Terragraph and the optical network in the KOIL Mobility Field, and the secondary connection is via a cellular network via the 4G/LTE router. For the wireless transmission experiment for sensing data, a 4K camera was placed, and the camera and Terragraph were supplied with signals and power via LAN cables from a power over Ethernet (PoE) switching hub. As a control computer, we used an aerial node device that was modified from the BUD device (2-core 1.8GHz Intel Atom CPU, Ubuntu 20.04, expansion processing module, and communication module) developed in the R&D item (2-d-i). The BUD device was designed for use both on the ground and in the air, and it was customized for use as a local node device for this study and then placed as a local computer. In addition, we installed a virtual desktop application (AnyDesk) to enable remote access from outside the network.
In the baseball field, we set up a similar system, which is a part of the KOIL Mobility Field. This enables the system developed at Fukuoka University to be quickly implemented and taken to the demonstration experiment phase. Figures 7–11 show an overview of the system in the baseball field. In particular, the Terragraph device (MLTG-CN) was provided with the same equipment that was installed in the KOIL Mobility Field. On the other hand, the BS unit (MLTG-360) was constructed as part of the Terragraph network provided by KOIL Mobility Field. The main unit also serves as a wireless BS for various ICWSN RF experiments.
The test field we have constructed here aims to enable the integrated management of ICWSNs that are widely distributed as a component of the proposed ICWSN framework. As shown in Figure 12, the test fields were set up at the KOIL Mobility Field (Kashiwa City, Chiba), the baseball field in the Nogata Office of Advantech (Nogata City, Fukuoka), and Fukuoka University (Fukuoka). These fields were interconnected via a broker placed on a cloud server provided by Sakura Internet and logically placed in the same segment via a virtual private network (VPN) connection. As a result, it can manage ICWSNs with wide-area distribution in a unified manner.
Each ICWSN test field consists of sensor nodes (SNs), relay nodes (RNs), and private base station (PBS). The RNs are further categorized as ground relay nodes (GRNs) and aerial relay nodes (ARNs). We deployed all of these key nodes in the field to provide an environment where we could conduct experiments. In addition, we assume that end users (data consumers) can obtain data directly by participating in ICWSN, and we have used Cefore, which enables ccnx-based communication, as a network platform for data retrieval.
The proposed ICWSN aims to integrate an air-to-ground network, and it is necessary to implement, develop, and evaluate an experimental testbed. Therefore, to provide the application services that underpin smart cities, we focused on how WSNs were an important element of the technology. We designed the wireless network to consider the communication environment, which is more extreme than wired networks, for example, signal attenuation due to radio wave propagation and fading caused by changes in the wireless communication environment. As an essential technology for the ICWSN to establish here, we investigated the fundamental characteristics of mmWave propagation in the NICT's anechoic chamber (Yokosuka, Kanagawa). The purpose of this evaluation is to determine the communication quality of Terragraph's beamforming technology and the propagation of waves, which is similar to optical waves when the transmitter (TX) and receiver (RX) are physically unstable (especially when the UAV is in motion).
As the hardware equipment for the communication system in the Terragraph, we used the master station (MLTG-360) and slave station (MLTG-CN), making up the mmWaves mesh network. According to the catalog specifications, the equivalent isotropic radiation power (EIRP) is 43 dBm for the master node and 38 dBm for the slave node. In the experiment, the communication distance between the master and slave nodes is 300m under the condition that the wireless link has a sufficient line of sight. The antennas on the master and slave Terragraph nodes are composed of 64-element phased arrays, with antenna gains of 28 dBi and 22 dBi, respectively, and beamforming acceptance angles of ±45° in the horizontal plane (azimuth) (φ) and ±25° in the vertical plane (elevation) (θ). The beamforming of the antenna is automatically set when the power is turned on, or the link is disconnected, based on the index pattern that indicates the direction of the pre-set antenna beam. In addition, there are four channels (center frequencies: 58.32, 60.48, 62.64, 64.80 GHz, bandwidth: 2.16 GHz) available in the 60 GHz band assigned to Terragraph communications.
Figure 13 shows the layout and construction of the experimental equipment. For the experiment, the master and slave nodes were placed 6.9m apart and positioned opposite each other as the initial position (i.e., the horizontal plane and direction of the radio waves were adjusted). We measured the throughput of the TCP and ICN layers using iPerf3 and Cefore as network and application characteristics. Figure 14 shows the average of the three measurements taken as the experimental results. In addition, the antenna power of the master and slave nodes can be obtained from the status information that can be seen on the management console, which includes EIRP (i.e., the transmission signal strength, which takes into account the transmission power, antenna gain, and feed attenuation) and RX-side RSSI (i.e., the received signal strength). The TCP throughput was stable at all angles, while the ICN throughput was stable in the 60° and 5° regions. We found that, although it is necessary to be careful that the antenna beam is narrower in the vertical direction than in the horizontal direction due to the specifications of Terragraph, it can tolerate some mobility when used as ARN and GRN.
Ⅳ.C. (2-d-ⅲ) Establishment of elemental technology for improving the reliability of ICWSNs (FY2023)
Here, we aim to establish the elemental technology for improving the reliability of data collection using ICWSN. Regarding the fundamental design of reliable data caching technology, we focused on removing the factors that prevent self-growth by solving the psychological barriers to joining the ICWSN as a zero-touch technology. As a result, we can contribute to promoting that growth by removing one of the factors that prevent self-growth. Specifically, we proposed a zero-touch functionality that focused on the lower layers, which is necessary for the initial operation of SNs when they join the ICWSN. In particular, in wireless network communication systems such as B5G, various devices are connected via multiple networks, and the users who have various backgrounds and motivations are connected, rather than simply scaling up with the addition or removal of random nodes. To achieve reliable communications, it is necessary to carefully consider the initial registration protocol to ensure that the data generated by the SN is kept clean (including the prevention of malicious data and the prevention of erroneous data due to equipment failure or malfunction, even if there is no malicious intention). Considering the above aspects, we first investigated how to ensure the reliability of the SNs that participate in the ICWSN. We have surveyed research and studies on design policies and related research for ICWSN, and we have also provided an overview of the technical issues that we would like to address through this. These were described as a blueprint.
We aim to improve the reliability of data collection for the ICWSN framework constructed in the R&D items (2-d-i) and (ii). As a fundamental study to accomplish the goal of the R&D item (2-d-iii), we designed an ICWSN system with zero-touch design that can be used for actual smart-city application services. In this section, we define high reliability as a benchmark that indicates that a system operates correctly over a certain time interval, i.e., a reliable system that can tolerate any errors that may occur (fault-tolerant).
As shown in Figs. 15–16, the proposed scheme uses a micro-operator (μO) to achieve a reliable system. In the proposed scheme, we consider a mechanism in which a network node can participate only when the μO verifies each message when the node is turned on. In order to reduce the initialization effort required when implementing the system in an actual smart city, it is necessary first to enable automatic registration using ICWSN. Therefore, for zero-touch design, the μO will perform its operations to manage device information. The information managed by μO can be shared between ICWSN, and the proposed method uses a blockchain-based ledger, an autonomous, decentralized network with no mutual trust. In ICWSN, it is generally difficult to support blockchain networks because the network nodes are limited in resources. The proposed method provides a solution in that it guarantees the reliability of nodes in the initial process by adopting the μO. Using the proposed method, the data reliability is guaranteed, and the data generated by a trusted node can be regarded as reliable without the need for additional verification. With data storage using blockchain, it is no longer necessary to undertake the computationally intensive mining that was previously required, and blockchain also has the advantage that it is not necessary to use the conventional consensus method, simply selecting an alternative consensus method. For example, there are proof-of-authority (PoA) and proof-of-elapsed-time (PoET).
In order to improve availability, the proposed scheme uses a proxy caching scheme. The proposed method can transfer the role of response to sensing data from relatively unreliable nodes (e.g., SN) to more reliable nodes (e.g., RN or PBS), and it can contribute to reliability improvement through cooperative data management. Based on the above studies, we investigated how to improve and expand the ICWSN system by emphasizing reliability and availability. In addition, since the proposed scheme has the potential to become an elemental technology that can solve other social problems (such as making cities greener, including carbon neutrality), the need to continue to consider this is also necessary.
As numerical results, we evaluated the conditions where Fig. 17(a) SN is less reliable than RN and PBS, (b) PBS and RN are equally reliable, and (c) PBS is more reliable than SN and RN. In the proposed scheme, using a proxy caching method, the overall availability can be improved by using a reliable node instead of a low-reliability node. Compared with the conventional method, the proxy caching technique is not used, and the proposed scheme can remove the issue that PBS is a single point of failure in the ICWSN system. The numerical results show that the proposed method improves availability, but even if we pay a great deal of cost to develop highly reliable hardware devices and make PBS overly reliable, it does not significantly improve overall reliability.
Ⅳ.D. (2-d-ⅳ) Cross-sectional demonstration and evaluation of integrated non-terrestrial ICWSNs (FY2024)
Developing a high-frequency spectrum to support next-generation wireless communication systems is an urgent task, and the mm-wave band communication used in this study (Terragraph is used in this study) contributes to the solution to this challenge. In the R&D item (2-d-ii), we conducted a fundamental evaluation of the integrated air-to-ground ICWSN using an ARN, and we also conducted a baseline evaluation for radio propagation measurements and long-term operation tests of mmWaves. In this section, we will evaluate the effects of weather (rainfall and snowfall) and the local environment on the wireless communication system by carrying out a long-term test operation, which was conducted as a test operation in the R&D item (2-d-ii). Regarding the realistic effects that cannot be eliminated when the system is actually deployed in a smart city, we will identify the issues that cannot be known from paperwork. Then, we will consider how to solve them. With the aim of improving the reliability of the ICWSN system under development, we intend to continue the current test operation and evaluate the impact of weather and the local environment on the propagation of radio waves. In addition, we will evaluate the applicability of the non-terrestrial ICWSN system from a variety of perspectives, with a view to its cross-development. In view of the cross-sectional development of the ICWSN system, we will discuss the implementation of characteristic evaluations assuming various communication environments, such as the characteristic evaluation of multiple aerial nodes, in the R&D item (3-b-①-iii).
Using the test field constructed in the R&D item (2-d-ii), we conducted an experiment between terrestrial nodes (PBS and GRN). This was a long-term operational test conducted as a preliminary phase before deploying the ICWSN system in an actual city. As an indicator of performance evaluation, we used iPerf3 to measure the TCP throughput (network bandwidth) of the wireless transmission link (Terragraph) between the PBS and GRN located in the baseball field. For periodic measurements, we implemented control software using Perl and designed it to enable easier reprogramming and reconfiguration with a user-friendly panel and operability using Node-RED. This was achieved through a policy of making it possible for anyone to install and operate the proposal system without specialized knowledge (zero-touch design). In the experimental network, the PBS and GRN were connected via point-to-point (P2P) connections with static local IP addresses assigned beforehand, and they were configured on the same local subnet.
Figure 20 shows the experimental results. The throughput was measured at 00 and 30 minutes (every hour), and the average of 1,440 (=48×30) measurement values were used as the daily average TCP throughput. The proof-of-concept experiment was conducted from December 19, 2023 to April 26, 2024. The average throughput for the entire experiment period was 940 Mbit/s, reaching the theoretical limit of 1 Gbit/s for Terragraph. Note that, although the IEEE 802.11 ad/ay specification defines a physical-layer data rate up to 1.93–4.62 Gbit/s, the wired LAN interface of the Terragraph equipment was compliant with Gigabit Ethernet, so this was a bottleneck. In addition, mmWaves are affected by weather conditions (rain). According to the Japan Meteorological Agency's rainfall data, during the 130-day experiment period, there were 66 days when it rained more than 1mm per day, and 18 days when it rained more than 10mm. In addition, the maximum rainfall was 19.5 mm/hr. and the average was 3.42 mm/hr. The results indicate that the weather conditions mentioned above did not significantly affect the experimental results (network characteristics).
During the experimental period, there were two incidents where measurement data could not be obtained: from February 2 to February 13 (11 days) in 2024, and from April 6 to April 26 (24 days) in 2024. This failure was not caused by Terragraph communication but was mainly due to the hardware and software of the measurement computer. For this network failure, if we classify it as a minor problem that can be solved by simply restarting the control computer or as a serious problem that requires dispatching a technician to investigate the cause and resolve the problem, this case was a minor problem. As a result, we found that the availability of the overall system can be improved by implementing a periodic diagnostic mechanism for the wireless links and devices in the proposed system and by implementing a mechanism for automatic recovery through automatic restarting in the event of a failure, with multiple nodes cooperating to monitor each other.
Using the test field constructed in R&D item (2-d-ii), we conducted an experiment between ground-to-air nodes (PBS-ARN) as a feasibility demonstration of an integrated ground-to-air ICWSN system. In particular, we implemented an ARN device and evaluated the characteristics between ground and aerial stations based on mmWave communications. Because of the limitations of the equipment (2–3 kg), the ARN used an industrial drone, and the system was assembled with an industrial computer (Advantech BUD), camera, and Terragraph node. The on-board computer on the UAV is part of the aerial node that was investigated in the R&D item (2-d-i). The camera and the Terregraph were connected to the computer via USB and Ethernet (LAN) cables, respectively. We used a PC (Intel Core i5U (2 core 1.3 GHz) CPU, 8 GB RAM, Ubuntu 20.04 OS) directly connected to PBS as the user terminal. Due to radio law and civil aeronautics law regulations, the UAV was moored in flight, and the rope was bundled with a LAN cable that supplied power to the Terragraph via PoE. After the UAV takes off, the ARN hovered at a height of = 5 m. The PBS (Terragraph master station) and ARN (Terragraph slave station) were positioned face-to-face, and the beamforming direction (index) was fixed by restarting the Terragraph slave station. Figure 21 shows the scene of the experiment in the field.
As for the network performance, TCP throughput was measured using iPerf3 at 30-second (1-second intervals). When the ARN hovered at positions with = 10, 20, and 30 m, the average throughput was 735, 787, and 899 Mbit/s, respectively. In the Terragraph stations, both nodes were placed at a distance of = 20 m. Compared to terrestrial communication, the average TCP throughput for non-terrestrial communication was 16.3% worse. In general, wireless communication quality degrades in proportion to the distance between the transmitter and receiver. However, in this experiment, the throughput for = 30m was stable compared to the results for = 10 m or = 20 m. Specifically, the standard deviations at = 10, 20, and 30 m were 245, 171, and 31.6, respectively, based on the statistical analysis of the throughput results. For this reason, if the UAV is maintained in a hovering status, the mmWave propagation characteristics are affected due to the up-down, left-right, and rolling-pitching motions. Therefore, as the distance between the PBS and ARN becomes longer, the effect of the UAV's fluctuations becomes smaller, and this contributed to the stability of the mmWave communication, as it has a strong straight-line directionality. In particular, since Terragraph equipment is used for fixed wireless mesh networks for terrestrial deployment, it is necessary to supplement the fact that beamforming is not dynamically (adaptively) reconfigured and that the antenna beam pattern is maintained while the adaptive modulation transmission rate (modulation and coding scheme (MCS) set) is adjusted down. The transmission power is increased in transmission power control (TPC) to maintain the wireless transmission link. The communication distance between the Terragraph master and slave units is 150m at maximum, so the communication performance is considered sufficient.
In order to evaluate the stability of mmWaves when the UAV moves up, down, left, and right, we measured the network performance with the UAV hovering at a distance of = 30 m, and then moved it 1m in each axis. As a result, the average TCP throughput was 931, 836, and 821 Mbit/s for hovering, vertical movement, and horizontal movement, respectively. In other words, the TCP throughput decreased up to 460 Mbit/s compared to the hovering status and decreased by 10.2% and 11.8% for vertical and horizontal motion, respectively. In addition, as the UAV moved, the fluctuations in the measured individual throughput values (at 1-second intervals) became larger, and the standard deviations for the hovering state, vertical movement, and horizontal movement were 31.6, 157, and 165, respectively. Although the TCP throughput temporarily decreased due to the UAV's movement, the transmission speed and wireless link were sufficient to maintain close to the throughput limit.
TCP throughput was measured for each radio section of BS–ARN and RN–SN. In the PBS–ARN section, the UAV was made to hover at points 10m, 20m, and 30m from the PBS. On the other hand, in the PBS&ndash ARN, the UAV hovered 20 m away from PBS, and it stayed over the SN. The TCP throughput is plotted as the result of a 30-second measurement using iPerf3. The average TCP throughput was 700–900 Mbit/s, which is close to the upper limit of Terragraph of 1 Gbit/s, and we could perform high-speed wireless transmission steadily. The results of the PBS&ndash ARN showed that the shorter the distance between PBS–ARNs, the greater the variation in throughput. Although the UAV is hovering, it is moving up and down, left and right, and also rolling and pitching, and this movement is affecting the propagation of the mmWaves. Therefore, the longer the distance between the PBS and ARN, the smaller the effect of such movement.
The ICN throughput is calculated as the average of the five measurements taken for each of the three different data sets. In the first data acquisition, the data was obtained from the SN via ARN, so the ICN throughput was lower. On the other hand, the average throughput from the second to the fifth time was improved compared to the first time due to the caching mechanism. The ICN throughput was calculated as an average based on the time taken to commit different random data (3 cases) to ICWSN using cefputfile and receive them using cefgetfile. In this case, when = 10, 20, and 30 m, the average ICN throughput was 12.2, 13.0, and 14.6 Mbit/s, and the average jitter was 712, 669, and 583μs. The characteristics of ICN throughput and TCP throughput indicated the same tendency.
We conducted an experiment in which live camera video streaming from the ARN was sent to a ground station, assuming a use case such as a disaster information-sharing system. Specifically, ARN was broadcast using cefputstream, and the PC connected to PBS received it using cefgetstream. Figure 22 shows the results of the experimental evaluation. There were no major problems with video streaming, but there was still the issue of video streaming not being smooth.
A testbed for an integrated non-terrestrial ICWSN, including an experiment with a ground-to-air node via mmWaves, was exhibited at the Japan Drone 2025 exhibition in Makuhari Messe (Chiba)(Figure 23). The demonstration was carried out using the Terragraph mmWave system, which we set up at the venue, and involved ICN-based real-time video streaming over a two-hop wireless link. Although the communication distance was short, it was confirmed that the system could continuously work stably during the exhibition period (3 days/10:00–17:00).
The zero-touch node developed here was used in a mmWave experiment in an actual city, with the cooperation of Nogata City, Fukuoka. It is necessary to consider the additional attenuation specific to mmWave, such as rain, oxygen, and hydrophilic substances (e.g., trees, leaves, and humans), in the link budget compared to the currently widely used radio frequency bands. Note that the 60-GHz band is sensitive to rain and oxygen. As related to studies, the application of mmWave communications for outdoor coverage within a few hundred meters is under consideration, but further evaluation is necessary to establish an ecosystem in an actual city. There have been few experiments on long-distance data transmission using mmWave communication in actual cities, and we believe that our contribution here is meaningful.
Here, we will describe the experimental environment. As shown in Fig. 24, the ICWSN nodes that have been implemented were placed in community centers and elementary schools in Nogata City, Fukuoka. The community center and elementary school are three-story buildings, and the node device was placed on the roof. According to the 3D map provided by the Geospatial Information Authority of Japan, the altitude of these buildings is 7.5 m and 16 m, respectively, and the straight-line distance between them is 1km. As shown in Figure 25, the wireless communication link passes through rivers, bridges, roads, and parking lots, which may affect radio wave propagation. The river is the Onga River, and the riverbanks are well-maintained and covered in grass and aquatic plants. During the experiment, the surface of the river was flat and calm, with no large waves; in other words, no factors affecting mmWave propagation were observed. The bridge crossing the river is Kanroku Bridge, and it connects to a local national highway. Nogata is a suburban city that continues to develop as a major city, such as Fukuoka and Kitakyushu, but the traffic flow on its main roads is not at a level that would affect mmWaves. The riverside area in front of the community center is used as a car park, and several dozen cars were parked here. Figure 26 shows the scene of the community center and school as an overview of the field where the long-distance wireless transmission experiment was conducted. The node devices that construct the ICWSN are connected to Terragraph equipment.
The two MLTG-CN/LR units (long-distance transmission models with built-in parabolic antenna) used here were facing each other, and according to the catalog specifications, the maximum distance over which communication was available was 1 km. During the experiment, the index for adaptive rate control was automatically set to 9. As shown in Fig. 27, taken from behind the antenna on the school roof, facing the community center; the community center is located at the position marked in red. As shown in this photograph, there is a clear line of sight between the transmitting and receiving nodes. In addition, the weather during the experiment was cloudy, so there was no effect on the propagation of mmWave radio waves due to rainfall attenuation.
The following two cases were assumed to be experimental scenarios. Namely, they are the case where the antenna surface is perfectly aligned (both elevation and azimuth angles are adjusted appropriately) and the case where the angle is slightly shifted from that state. In the MLTG-CN/LR device, the space in front of the antenna is divided into a grid pattern from the elevation angle and azimuth angle, and the beamforming index is assigned to each sub-area. In addition, the beam direction closest to the center of the antenna surface has a beamforming index of 30, which confirms that the results of adjusting both antenna surfaces are the same for both scenarios.
Figure 28 shows the experiment results to evaluate network and ICN performance. The average TCP throughput between the antenna surfaces and when not aligned was 941 Mbit/s and 94.4 Mbit/s, respectively. The antenna integrated inside the MLTG-CN/LR is a parabolic antenna with directional characteristics, so a few degrees of misalignment of the antenna surface caused a degradation in network characteristics. Regarding TCP congestion control, the average window size was 1.26 Mbytes and 0.967 Mbytes, with a difference of 39.3% observed. When the antenna surfaces are matched, the results show that the size of the congestion window is increased.
The average UDP throughput was 902 Mbit/s and 93.3 Mbit/s, respectively. When the antenna surfaces were matched, there was a temporary drop in UDP throughput. The cause of this degradation is because of the automatic retransmission request and forwarding error control mechanisms. Note that, compared to the TCP features, this type of degradation is not seen because the TCP congestion control mechanism is working effectively. The average packet error probabilities of UDP communication were 0.0294 and 0.903, respectively. When the antenna surfaces are mismatched, packet loss is irregularly observed, and it affects UDP throughput. In addition, the average ICN throughput was 16.1 Mbit/s and 15.8 Mbit/s, respectively.
The ICN throughput was significantly smaller than the TCP and UDP throughputs because of the bottleneck in Cefore. Note that the ICN layer is stacked above the TCP/UDP layer in ICWSN. Therefore, when the maximum data bandwidth is set to the maximum value due to the implementation of middleware in Cefore, the probability of failure in data registration, storage, and transfer is worsened. In addition, the average jitter was 525μs and 534μs, respectively. The two scenarios had no significant difference in ICN throughput and jitter. From these results, it was found that the performance of the ICN layer protocol does not affect the performance of the TCP/UDP/IP protocol stack. We also experimentally verified that sufficient network performance can be achieved with ICWSN using mmWaves in the actual city.
Ⅳ.E. (2-d-ⅴ) Demonstration and evaluation of reliable ICWSNs (FY2024)
Using the expertise we have acquired in the R&D items (2-d-i) to (iv), we aim to develop a reliable zero-touch sensor node and to evaluate the effectiveness of the proposed method experimentally by conducting operations in two locations: the KOIL Mobility Field and the area around Fukuoka University.
In the R&D item (2-d-iii), we investigated a method that uses micro operators (μO) and micro service providers (μSP) to achieve high reliability and zero-touch design in the proposed ICWSN. The μO verifies whether SN can join ICWSN, and it provides the information necessary for the connection based on the verification results. Therefore, it is necessary to exchange not only authentication information but also network configuration information (i.e., the information on the nearest RN, PBS, and forwarding information base (FIB)). On the other hand, μSP provides the applications and configuration information necessary for zero-touch operation after the SN has established a connection with ICWSN. Using the knowledge of reliability engineering in wireless networks, we formulated the availability of the proposed method and evaluated it through desk-based studies. In the R&D item (2-d-iii), the design and analysis were completed on paper, but there are still some insufficient points when considering actual implementation. At this point, it is necessary to continuously conduct further studies. As the first step, developing a prototype node and conducting a fundamental evaluation is necessary.
As for the development of zero-touch node, through R&D items (2-d-i) to (iv), we developed a portable node device with various sensors and peripheral devices in an attaché case to conduct various experiments in the demonstration field. In addition, we have developed a node and a locally placed node device that can be used on the ground and in the air. By integrating this knowledge, we developed a reliable zero-touch node device. The nodes are waterproof and designed to be placed in extreme outdoor environments, and the waterproof connectors are used on the inside and outside of the device. To improve the reliability, the developed system was not equipped with a mechanical structure, such as a motor-driven system or air-cooled fans. The sensor and various modules were designed to be mounted outside the case, and their interfaces are standardized as serial connections based on RS-232/485 (Modbus), which is a de facto standard in the industrial field, making it a general-purpose design with compatibility and expandability. Since this device uses a zero-touch design, the settings are pre-configured to work simply by pushing the power button after connecting to a commercial power supply. The zero-touch node device was exhibited as a prototype at CEATEC 2024 (Fig. 29).
It is pointed out that the zero-touch node device developed here requires ICN-based data transmission control and must have sufficient specifications. We modeled sensing data in smart-city applications based on their statistical characteristics to evaluate this perspective. We evaluated the computational processing power of the control computer assumed as a constituent node of the ICWSN for the random data generated using the formulated model.
We formulated a statistical model of sensing data using data acquired by the river monitoring system developed in the previous study for disaster-resistant smart cities. To prevent internal flooding caused by typhoons and heavy rainfall, the trial system was placed on the Onga River (Nogata City, Fukuoka) to predict the flow speed and direction of the river as a river monitoring system. The sensing data are then image data of photographs of the water surface and surrounding landscape. For the statistical modeling here, the data set contains 25 pairs of 20 images with the same conditions, such as position, camera angle, and shooting time. In the specific procedure for creating the model, we calculated a statistical model of the sensing data that represents the cumulative distribution function (CDF) of codewords (0x00 to 0xFF) in bytes from the data set.
The frequencies of 256 different code words were calculated for each data set, and the probabilities of occurrence were sorted in descending order. Figure 30 shows the result of plotting the computed CDF as a superimposed line. The red line represents the case where the code words occur equally. The results indicate that the sensing data obtained in the field has a specific bias; the line should overlap the red line if the code words are evenly distributed. Although the conditions of acquisition were different, the statistical characteristics of the data were similar, and therefore, this statistical model was used to generate random data to evaluate hardware performance.
Since sensing data are managed as encapsulated named data in ICWSN, the data are compressed and encrypted as fundamental data calculations to ensure efficiency and reliability. Thus, we evaluated the hardware devices assumed as user terminal nodes, edge nodes, and SNs as nodes on the edge side of the proposed ICWSN in terms of their computational power. The results of this evaluation will contribute towards providing benchmarks and achievable conditions for establishing and deploying networks in smart cities. For hardware, MacBook Pro was used as the user terminal node, Advantech AIR-020 as the edge node, and an Advantech EPC-S202 and Raspberry Pi 4B as SNs. In particular, the Advantech AIR-020 and EPC-S202 are highly reliable industrial applications embedded computers. To evaluate the computing capacity of the computer, we prepared 100 random data sets of 0.1, 0.5, 1, 5, 10, 50, 100, 500, 1,000, 5,000, and 10,000 kbytes, which were generated according to the aforementioned model. As a variation of the dataset, the minimum and maximum sizes were set, assuming text-based sensing data with ICN headers (and footers) and typical ICN chunk sizes. The purpose of this procedure for data generation was also to avoid the use of actual data, which could leave concerns about issues related to copyright and portrait rights.
The processing time for data compression and decompression processing was determined during the experiment. Specifically, the deflate algorithm and the Lempel-Ziv-Markov-Chain algorithm (LZMA) were used as data compression algorithms. The deflate method uses a sliding dictionary and Huffman coding technology and is a widely used data compression method. The LZMA method is one of the most efficient data compression methods, and it is enhanced with various coding techniques to improve compression performance. Both are lossless data compression methods and are supported by several embedded devices. In general, the LZMA method is faster than the deflate method. The AES method, a symmetric key cryptosystem widely used in various computer systems, was used as a data encryption method. In general, in AES-based systems, the key length is selected as 128-bit, 192-bit, and 256-bit. The longer the key length, the stronger the encryption strength. Here, we selected 128-bit and 256-bit as they are widely used in common IoT devices. In the experiment, we used OpenSSL, an open-source software library that provides secure communication over Internet servers, such as the HTTP over TLS/SSL protocol.
In Figs. 31–32, the results for data compression include the processing times for each of the deflate and LZMA methods, and for data encryption, the results include the processing times for encryption and decryption using the 128-bit and 256-bit AES methods. Note that both the vertical and horizontal axes are logarithmic. In the region where data size is less than 100 kbytes, the data compression/decompression processing time is almost the same, and both deflate and LZMA methods process up to 100 kbytes in less than 10 ms, regardless of the device. It represents the minimum processing delay required for data (compression), including processing overhead like initialization and termination, which is unavoidable. On the other hand, in the region over 100 kbytes , the processing time increased in proportion to the data size. Data encryption processing time shows the same trend as data compression processing time. MacBook performed better than the other devices. Although the AIR-020 is built on the Jetson platform, the AI optimization mechanism did not work effectively for general-purpose processes like data compression and encryption. The MacBooks had the best performance of the devices tested because of their powerful end-user processors. On the other hand, WSN devices on the edge need to be considered for outdoor use, and due to heat dissipation and stability issues, the processing delay is a compromise specification of approximately 100 ms.
We discuss the effect on the deployment of ICWSN in smart city applications for the results in Fig. 31–32. The edge-side node device's processing delay was less than 100 ms. Namely, as a result, application services for which latency is not a major issue or applications that are not latency sensitive, e.g., those with a data collection interval of one hour (or one day), will not be a concern. River monitoring systems for disaster-resilient smart city applications require sensing data every hour under normal weather conditions. However, this interval may be reduced to 20 minutes or less in a disaster; this is not a concern.
We tried a different approach to achieve wireless transmission with cashing data. The network layer of ICWSN is the same broadcast-based data transfer as wireless communication (unlike wired networks). Although this means that ICWSN is a suitable combination for wireless communication, radio wave overhearing occurs in broadcast-based wireless transmission. In order to overcome this disadvantage, we have investigated cooperative communications based on constructive interference and network coding (NC) technology. This scheme adds an assist phase after data transmission, and cooperative nodes around the source node send extra data to gain diversity and advantage. Here, we conducted initial investigations into a wireless transmission method that directs new data caching, aiming to eliminate the assist phase by utilizing hierarchical modulation (HM) technology.
In wireless communication systems, it is generally designed to combine different modulation methods and error correction coding methods to achieve flexible data rates. In addition, in the case of wireless digital TV broadcasting systems, superimposing multiple information bits using HM technology achieves a certain degree of flexibility while maintaining backward compatibility with fixed data rate receiving devices. We noticed that the ICWSN and TV broadcasting systems have the same broadcast-based characteristics, and we have developed a method that can integrate two different information sources by expanding the concept of HM technology. Here, we came up with eliminating unnecessary data transmission during the assist phase. As an initial investigation, we present a blueprint for a cooperative communication method based on HM technology and evaluate its fundamental characteristics as a preliminary evaluation.
In a wireless communication system that uses HM technology, as shown in Figure 33, multiple types of information are classified into primary and secondary information, which are superimposed. In the proposed scheme, the original data is assigned to the primary information, and the data necessary for a cooperative node is assigned to the secondary information. These information signals can be modulated and superimposed using the QPSK method, which is commonly used in digital wireless communications, to generate signals similar to those of the 16-QAM method. The hierarchical QPSK/16-QAM method used in the proposed method has a different information mapping rule from the usual method; primary information is assigned to the upper two bits of 16-QAM, and secondary information is assigned to the lower two bits. As a result, the receiver node can separate the primary and secondary information if it can perform the HM technique. On the other hand, the nodes that do not support the HM technology can still maintain compatibility because it is possible to restore the primary information according to the QPSK method.
Figure 34 shows the results of the computer simulation evaluation conducted as a fundamental evaluation of the proposed method. Figure 34(a) shows the ratio of the coverage area of the primary and secondary information that can be received for the main parameters of the HM technique. As shown in Fig. 34(b), the number of cooperative nodes by the proposed method increases as the number of nodes increases, but the ratio to the nodes within the communication range remains constant. Figure 34(c) shows that if the nodes that can use HM technology coexist at a certain rate, the number of cooperative nodes increases exponentially. Figure 34(d) shows that it increases in a linear manner under some variable factors. As the results of these initial simulations, it is necessary to clarify the conditions under which the proposed scheme can work effectively, and this requires the continuation of similar studies in the future and the design of a system based on the results.
Ⅳ.F. (3-b-①-ⅰ) Prototyping: 3D sensor system (fundamental design)(FY2022)
We have implemented a prototype based on the MIC-710AIX from Advantech, which has a well-established history as an embedded computer for industrial use. We developed a portable node in the R&D item (2-d-i). Regarding constructing a prototype network environment and implementing fundamental evaluation, we conducted a preliminary evaluation of the feasibility of mmWave applications in ICWSN using the test device implemented in the R&D item (2-d-i). Specifically, we assumed point-to-point communication between user terminals and sensor nodes, and the user terminal was a MacBook and Terragraph base station, whereas the sensor node was a prototype device from the R&D item (2-d-i). As measurement metrics, we measured TCP throughput using iPerf3 and ICN throughput on the Cefore ICN platform. Regarding the measurement of ICN throughput, we carried out the application-level verification that we had been advised to do at the start-up meeting. Through the fundamental evaluation, it was found that the propagation properties of mmWaves are significantly affected by rain, trees and leaves, and human beings blocking wireless transmission links, rather than distance attenuation and that the throughput is reduced by up to 50% just by a human crossing during the experiment. In addition, we found that sufficient throughput can be achieved for wireless transmission of 3D sensing data (Figs. 35–37).
In the experiments conducted here, when the transmission link between the Terragraph transceivers was measured without any consideration for the horizontal plane, the throughput decreased by 10%. In the Terragraph, we thought that the direction of the antenna in the horizontal plane could be adjusted due to the operation of the beamforming technology, but it became clear that it was necessary to investigate the impact of the throughput decrease due to the elevation angle. In the aforementioned essential evaluation, experiments were conducted using a laser rangefinder to maintain the horizontal plane and the distance between the antennas. Considering the above, we evaluated the effect of throughput degradation due to changes in elevation angle in an anechoic chamber (an environment where the effects of reflected waves are eliminated). Specifically, we carried out communication tests between the master and slave units of Terragraph, throughput measurements, and content transmission and receiving tests using Cefore. In this case, the throughput was measured when the antenna angle of the parent and child units of Terragraph was changed in 5° increments from 0°–15°, and the expected test results were obtained (Fig. 38). When considering the deployment of nodes in the sky or in smart cities, it was also found that it was necessary to evaluate the performance of beamforming further.
Ⅳ.G. (3-b-①-ⅱ) Prototyping: 3D sensor system (test operation)(FY2023)
A prototype network will be constructed and tested in a practical field. In association with the R&D item (2-d-ii), we implemented a system that allows for multidimensional operational testing not only using the KOIL Mobility Field but also the area around Fukuoka University. We constructed a prototype network in a physical field and evaluated the network performance in a ground-to-air integrated ICWSN environment. In this experiment, we experimented with the baseball field, and we implemented an aerial-node device, as shown in Fig. 39. The ARN device consisted of a customized BUD device, a camera, and two Terragraph devices, and they were mounted on an industrial unmanned aerial vehicle (UAV). Terragraph is used for communication between PBS and SN, so one Terragraph pair is used to connect PBS and ARN, and the other is used to connect ARN and SN. Therefore, the antenna was oriented towards the horizontal and ground planes.
Figure 40 shows our experimental network structure, and we used a PC directly connected to the PBS as the user terminal. We installed Cefore's ICN platform on the PC and the BUD device, and we specified the FIB to ensure that data is forwarded from the user terminal to the SN. Namely, we assigned different subnet masks to SN–RN and RN–PBS, and then set up the FIB table as the name and address mapping rule when relaying from PBS to SN via ARN. The ARN provides a built-in caching capacity in random access memory (RAM), and it can respond to requests for the same data.
Ⅳ.H. (3-b-①-ⅲ) Prototyping: 3D sensor system (proof-of-concept experiments with horizontal scalability)(FY2024)
Based on the test operation that was implemented in FY2023, we experimented in collaboration with other organizations for horizontal development. This experiment aims to investigate the reliability (stability) of wireless communication links in a multi-hop (3-hop in this experiment) environment as an experiment for integrated non-terrestrial ICWSNs. To carry out this verification, it is necessary to prepare multiple aerial nodes, so we evaluated the system using an environment that can simulate aerial nodes using the smart poles owned by the Shibaura Institute of Technology. Figure 41 shows the experimental network. A PC was connected to the Terragraph base station installed in the KOIL Mobility Field. The SN used a zero-touch node device developed in the R&D item (2-d-v) and connected a Terragraph as a wireless interface. As relay nodes in the sky, two sets of smart poles equipped with two Terragraph terminals each were prepared, and a demonstration experiment network was constructed with a total of three hops, linking the Terragraph master terminal and zero-touch node devices, and linking the relay nodes in the sky. Each device was assigned a fixed IP so they would be on the same network.
We assumed two scenarios for constructing the experimental network in the KOIL Mobility Field, as shown in Fig. 42–43. Namely, all nodes are placed in a straight line and bent vertically. We measured the fundamental network for these two scenarios. Figures 44–45 show the experimental scenes, including the installation of smart poles to simulate the upper-air nodes and the installation of sensor nodes. The TCP throughput was obtained using iPerf3, the same as the maximum throughput of Terragraph (1 Gbit/s). Based on these results, it was shown that the stable transmission of mmWave in a multi-hop environment via an aerial node is actually feasible.
Ⅴ. Conclusion
In these R&D items, we have developed a reliable data caching technology for ICWSNs, which is a WSN that uses an ICN as a technology for a self-growing digital twin.
Acknowledgement
This work was partly supported by NICT Japan, Grant Number JPJ012368C05601. We are grateful to Dr. Kenji Kanai and Dr. Taku Yamazaki for their helpful discussions, and to Nogata City, Advantech Japan, BeMap, Haft, Panasonic, and TEAD for their help with the experiments.
Research Outcomes
- 金井謙治, 山崎託, 宮田純子, 金光永煥, 峯荒夢, 森慎太郎, 中里秀則, “City as a Serviceを支えるデジタルツインを持続可能な状態で自己成長させるエコシステム,” 電子情報通信学会 コミュニケーションシステム(CS)研究会 技術報告, vol. 122, no. 269, pp. 1–6, 名古屋, Nov. 2022.
- Shintaro Mori, “A preliminary evaluation of millimeter-wave communications for information-centric wireless sensor networks,” Proc. 2023 RISP International Workshop on Nonlinear Circuits, Communications, and Signal Processing (NCSP 2023), p. 130, Honolulu, USA, Feb.–Mar. 2023.
- Shintaro Mori, “Information-centric wireless sensor networks for smart-city-as-a service: Concept proposal, testbed development, and fundamental evaluation,” Proc. IEEE Consumer Communications & Networking Conference (CCNC 2023), pp. 945–946, Las Vegas, USA, Jan. 2023, doi: 10.1109/CCNC51644.2023.10060577. (Xplore, Digital library)
- 森慎太郎, “(チュートリアル)グリーン情報指向無線センサネットワーク実現のための高効率・省電力化に関する一検討,” 電子情報通信学会 総合大会 2023, 埼玉, Mar. 2023.
- Shintaro Mori, “A study on zero-touch-design information-centric wireless sensor networks,” Proc. IARIA the 22th International Conference on Networks (ICN 2023), pp. 7–9, Venice, Italy, Apr. 2023. (Best paper award) (Thinkmind, Digital library)
- 金井謙治, 中里秀則, 山崎託, 宮田純子, 金光永煥, 峯荒夢, 森慎太郎, 今村博宣, “(依頼講演)D2EcoSys:共創型デジタルツインの実現に向けて,” 電子情報通信学会 コミュニケーションシステム(CS)研究会 技術報告, vol. 123, no. 32, p.26, 高松, May 2023.
- 森慎太郎, “(依頼講演)情報指向無線センサネットワークに関する一検討,” 電子情報通信学会 コミュニケーションシステム(CS)研究会 技術報告, vol. 123, no. 32, p. 33, 高松, May 2023.
- 森慎太郎, “高信頼情報指向無線センサネットワーク構築に向けた初期検討:プロトタイプネットワーク構築およびテストベッド開発,” 電子情報通信学会 情報指向ネットワーク技術(ICN)特別研究専門委員会, 福岡, Aug. 2023. (Slide decks)
- Shintaro Mori, “Energy-efficient cooperative caching scheme for green ICWSN: Preliminary analysis and testbed development,” Proc. ACM the 29th Annual International Conference on Mobile Computing And Networking (MobiCom 2023) WS Networked Sensing Systems for a Sustainable Society (NET4us), pp. 207–212, Madrid, Spain, Oct. 2023, doi: 10.1145/3615991.3616406. (ACM, Digital library)
- K. Kanai, H. Kanemitsu, T. Yamazaki, S. Mori, A. Mine, S. Miyata, H. Imamura, and H. Nakazato, “(Invited) D2EcoSys: Decentralized digital twin ecosystem empower co-creation city-level digital twins,” IEICE Transaction on Communications, vol. E107-B, no. 1, pp. 50–62, Jan. 2024, doi: 10.1587/transcom.2023WWI0001. (J-Stage, Digital library)
- Shintaro Mori, “Zero-touch-design information-centric wireless sensor networking with availability assurance,” International Journal on Advances in Networks and Services, vol. 16, no. 3&4, pp. 63–74, Dec. 2023. (ThinkMind, Digital library)
- Shintaro Mori, “Information-centric wireless sensor networking platform for decentralized and co-creative digital twin eco-system,” Proc. 2024 RISP International Workshop on Nonlinear Circuits, Communications, and Signal Processing (NCSP 2024), pp. 348–351, Honolulu, USA, Feb.–Mar. 2024.
- Shintaro Mori, “Test-field development for ICWSNs and preliminary evaluation for mmWave-band wireless communications,” Proc. IEEE Consumer Communications & Networking Conference (CCNC 2024), pp. 1–2, Las Vegas, USA, Jan. 2024, doi: 10.1109/CCNC51664.2024.10454799. (Xplore, Digital library)
- 森慎太郎, “ミリ波帯地対空情報指向無線ネットワークを用いた鳥瞰映像伝送に関する一検討,” 電子情報通信学会 情報指向ネットワーク技術(ICN)特別研究専門委員会, 伊勢, May 2024.[Slide decks]
- Shintaro Mori, “MmWave UAV-assisted information-centric wireless sensor network for disaster-resilient smart cities: Preliminary evaluation and demonstration,” Proc. IARIA the 23th International Conference on Networks (ICN 2024), pp. 1–4, Barcelona, Spain, May 2024. (Best paper award) (Thinkmind, Digital library)
- 森慎太郎, “(依頼講演)情報指向無線センサネットワークにおける高信頼性ゼロタッチノードの試作とその応用に関する一検討,” 電子情報通信学会 コミュニケーションシステム(CS)研究会 技術報告, vol. 124, no. 234, pp.20–25, 大阪, Nov. 2024.
- Shintaro Mori, “(Invited)A study on information-centric wireless sensor network framework for smart-city deployment,” IEICE SeMI Vietnam Workshop, Da Nang, Vietnam, Oct. 2024.[Press]
- Shintaro Mori, “Development of UAV-aided information-centric wireless sensor network platform in mmWaves for smart-city deployment,” International Journal on Advances in Networks and Services, vol. 17, no. 3&4, pp. 105–115, Dec. 2024. (ThinkMind, Digital library)
- Shintaro Mori, “Fundamental analysis of cooperative communication scheme using hierarchical modulation for information-centric wireless sensor networks,” Proc. IEEE Consumer Communications & Networking Conference (CCNC 2025), pp. XX–XX, Las Vegas, USA, Jan. 2025, doi: XXX. (Xplore, Digital library) (Accepted)